
The Challenge of PFAS in Drinking Water Supplies
What are PFAS and why are they a concern?
Per- and polyfluoroalkyl substances are a persistent group of manufactured organofluorine chemicals that have served many purposes in industrial and consumer product applications. Also referred to as perfluorinated compounds, PFAS have been utilized for their ability to repel oil and water and long-term stability. Several compounds are included in the PFAS family, including perfluorooctanoic acid and perfluorooctane sulfonate (Figure 1). These two compounds are the primary concern to drinking water utilities and regulators since they are present at increased concentrations in source waters and have public health implications.
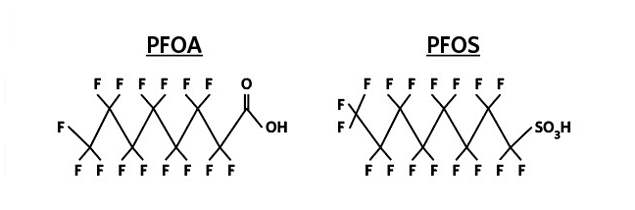
PFAS are very stable in the environment. Not only are they slow to degrade, but they can form degradation by-products that also present toxicity concerns. Their presence has been measured in water, soil, air, food and even human blood. The half-life of PFAS in the human body can range from four months to over nine years. Increased exposure to high concentrations of PFAS has been shown to cause negative health effects in laboratory animals, such as abnormal endocrine activity, tumors, organ damage, reduced immune system function and reproductive and developmental problems.
PFAS can be clustered into two primary groups: “long-chain” and “short-chain” (Figure 2). Long-chain PFAS typically are designated as perfluoroalkyl sulfonic acids containing six or more carbons, and perfluoroalkyl carboxylic acids containing eight or more carbons. Short-chain PFAS, which have fewer than six carbon molecules, can be degradation by-products of long-chain PFAS or independent compounds. Since degradation of long-chain PFAS is more challenging, they tend to persist in aquatic environments longer than short-chain PFAS. Conversely, short-chain PFAS can be more difficult to remove by treatment methods, like activated carbon, due to their limited capacity for adsorption.
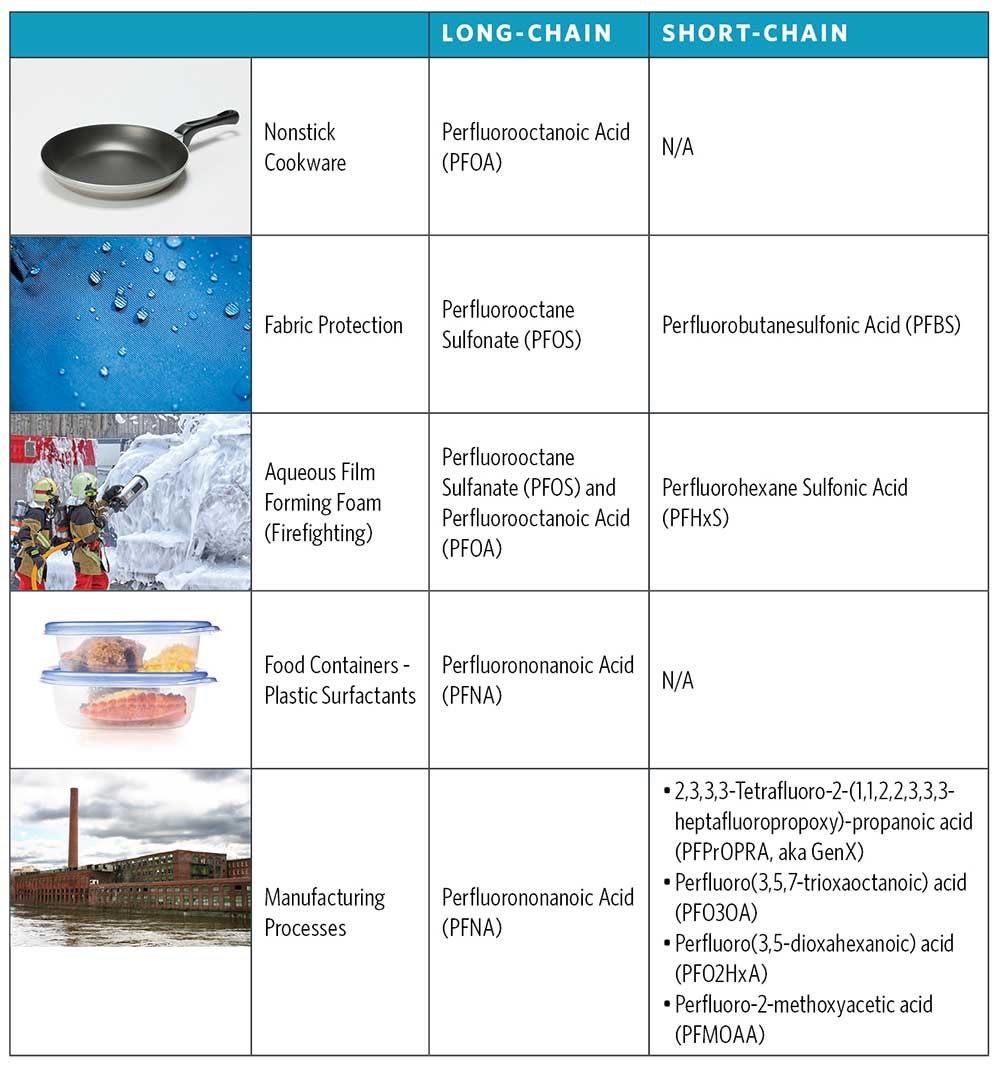
Regulation of PFAS
Presently, there are no enforceable federal drinking water limits for PFAS or any degradation products. As the negative health effects of PFAS became more apparent in the early 2000s, the U.S. Environmental Protection Agency began to provide PFAS concentration guidelines through provisional health advisories for PFOA and PFOS. In an effort to phase out usage of PFAS, EPA’s 2010/2015 PFOA Stewardship Program coordinated emissions reduction of PFAS with eight large chemical companies. Under this program, companies aimed to reduce 95 percent of PFOA and long-chain PFAS by 2010 and completely eliminate emission of long-chain PFAS by the end of 2015.
Independent studies conducted by the Department of Defense identified PFAS as a group of emerging contaminants because they have accessible pathways to enter the environment, pose a human health or environmental risk and do not have regulatory standards based on peer-reviewed research.
In May 2016, the EPA released drinking water lifetime health advisories for PFOA and PFOS that are based on adverse reproductive and developmental health effects. The new LHAs recommend that PFOS and PFOA concentrations, individually or combined, be limited to 70 (ng/L) to prevent potential public health impacts. In addition to the federal PFAS LHAs, individual states have adopted their own regulations and monitoring guidelines. New Jersey, Massachusetts, Michigan, New Hampshire, New York, and Vermont have developed effective maximum contaminant levels for PFAS. Some states have MCLs for individual PFAS, such as New Jersey (PFNA = 13 ng/L, PFOS = 13 ng/L, PFOA = 14 ng/L). Alternatively, some states have effective MCLs for the sum of multiple PFAS, including Vermont (PFOA + PFOS + PFNA + PFHxS + PFHpA = 20 ng/L). Other states, including Ohio, Connecticut, California, Rhode Island, Maine, Illinois, Texas, North Carolina, Minnesota, Pennsylvania and Washington have all developed guideline concentrations for PFAS in different water matrices. Some state guidelines focus primarily on groundwater remediation objectives, such as North Carolina (groundwater interim maximum allowable concentration of PFOA = 2,000 ng/L), while other states have focused on drinking water concentration guidelines, like Maine (drinking water guidance level for PFOA + PFOS = 70 ng/L).
Presence of PFAS in Drinking Water Supplies
PFAS are very soluble in aquatic systems, making them difficult to remove from various water sources. The chemical nature of PFAS makes biodegradation, photolysis and hydrolysis more challenging. PFAS can partition into water from various sources, such as consumer products, indoor and outdoor air, food packaging and other points of origin. The use of firefighting foams and industrial discharges typically provides the highest point source concentrations of PFAS in source waters. It is also possible for PFAS to enter drinking water sources such as groundwater or surface waters through wastewater effluent and application of biosolids, landfill leachate and stormwater runoff streams.
In the United States, PFAS have been identified in source water in the microgram per liter (µg/L) level or lower and finished water locations at the ng/L level. Data collected as part of the EPA’s Unregulated Contaminant Monitoring Rule 3 (UCMR 3) provides an assessment of PFAS presence in public water systems (PWSs). Results from sampling at 4,864 PWSs showed 0.9 percent of PWSs exceed the LHAs for PFOA and 0.3 percent of PWSs exceed the LHAs for PFOS. Other measured PFAS were below detection limits. However, there are a few limitations with PFAS results obtained through UCMR 3. Only select PWSs were tested for PFAS, and only six PFAS were measured (PFOA, PFOS, PFBS, PFHxS, PFHpA, and PFNA). Additionally, reporting limits during testing were relatively high (10 to 90 ng/L) compared to current analytical capabilities; therefore, PFAS reported as “not detected” could actually be present, but at levels below the UCMR reporting limits.
The Environmental Working Group (EWG) has an interactive map (Interactive Map: PFAS Contamination Crisis: New Data Show 2,337 Sites in 49 States (ewg.org)) to show the extent of PFAS detection throughout the United States. These sites include drinking water sources, military sites, and other known sites such as landfills or wastewater treatment plants.
Several states have taken the initiative to identify PFAS presence in drinking water supplies. For example, the State of Michigan established the Michigan PFAS Action Response Team (MPART) in 2017 to investigate sources and locations of PFAS in drinking water throughout the State. Additionally, the North Carolina Department of Environmental Quality and the Department of Health and Human Services have been investigating the presence of PFAS in water sources since 2017. This investigation led to the halt of PFAS release into the Cape Fear River.
Treatment Options for Removal of PFAS
The removal efficiency of treatment techniques for PFAS from source waters depends on influent water characteristics including the total PFAS concentration and the treatment method utilized. While conventional treatment methods have proven to be unsuccessful for PFAS removal, advanced treatment techniques can reduce PFAS concentrations by 90 percent or higher. Figure 4 shows PFAS removal rates for advanced treatment methods.
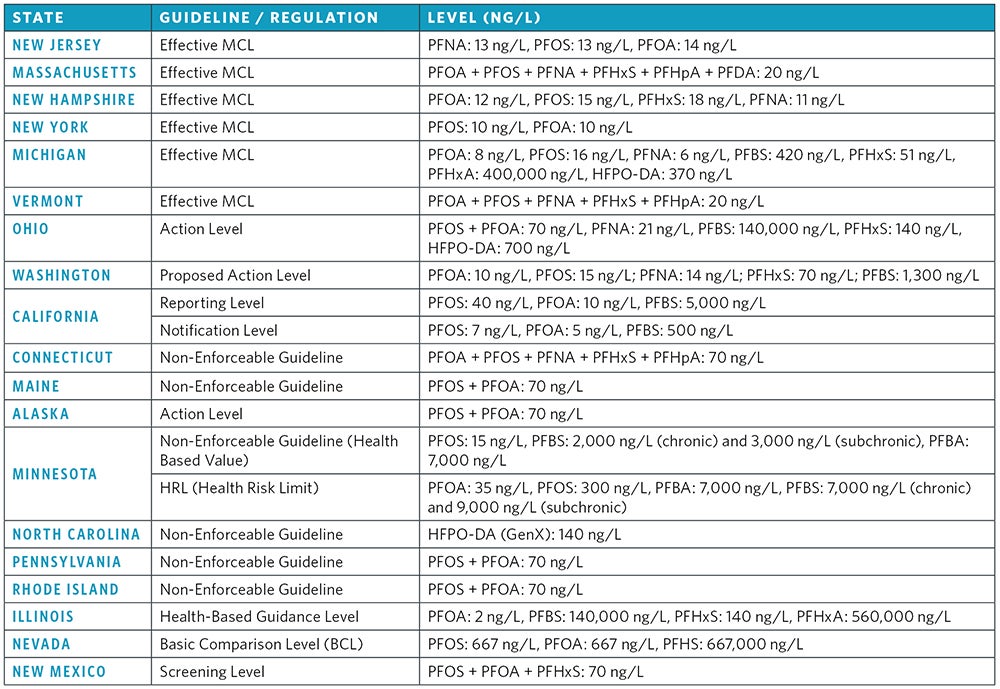
ACTIVATED CARBON ADSORPTION
Granular activated carbon and powdered activated carbon are used for removal of PFAS from source waters. GAC is a highly effective and commonly used treatment method for persistent long-chain PFAS removal with greater than 90 percent removal of PFNA, PFOA and PFOS. Process optimization may be necessary to ensure that pH, temperature, empty bed contact time and other water quality factors are optimal for effective removal of PFAS. Challenges with GAC are that PFAS removal will decrease as adsorption sites become exhausted with PFAS and other organics, requiring media replacement. PFAS will need to be removed from exhausted media and PFAS removal from media using regeneration is not yet fully understood.
PAC is another form of activated carbon with smaller diameter particles that can be added to as a pretreatment step at a water treatment plant. Higher concentrations of PAC may be necessary to reach the same removal rates as GAC, which can increase the costs for treating PFAS. Like GAC, PAC is more effective at long-chain PFAS removal compared to short-chain PFAS removal. The success of PFAS adsorption depends on a number of factors, including influent PFAS species and concentration, the presence of other water quality parameters, and system design. One major challenge with PAC is the impact on residuals handling. Residuals loading will be higher and will contain PFAS, which may not be accepted by a residuals handling facility.
ANION EXCHANGE
Anion exchange utilizes a special ion exchange material (usually commercial resins or petrochemical compounds) shaped as beads to exchange anions in untreated water and replace them with hydrogen or hydroxyl ions. The rate of exchange will depend on many factors, including influent PFAS concentration, other water quality parameters present, design of the anion exchange system and bead material. While anion exchange has shown varying degrees of effectiveness in removing PFAS, porous anion exchange resin impregnated with iron oxide has been shown to remove up to 90 percent of some PFAS from drinking water. Similarly to GAC, PFAS removal will decrease as IX resin sites become exhausted with PFAS and other water quality parameters, requiring resin replacement and disposal.
MEMBRANE SEPARATION
Reverse osmosis and nanofiltration are both effective methods for removing PFAS from drinking water. RO and NF membranes are a semi-permeable membranes that retain PFAS on the pressurized side of the membrane while the purified water passes through it. RO is most commonly used to remove dissolved salts, but organics are also removed using this process. RO works most efficiently when feed water is pretreated to prevent membrane fouling and scaling and has been shown to achieve up to 99 percent removal of PFOS and PFOA from drinking water.
NF is similar to RO, but with smaller membrane pore sizes (around 0.001 micron). NF membranes operate with a higher water flux to remove many contaminants, including organic molecules, viruses and divalent salts. NF has been shown to remove up to 90 percent of PFOS and PFOA from drinking water. RO and NF membranes produce a concentrate stream containing rejected constituents. This is a major challenge in PFAS applications because this reject stream contains PFAS and should be properly disposed of to reduce contamination into nearby waters.
ADVANCED OXIDATION
Advanced oxidation processes utilize strong oxidants such as UV/H2O2, Fenton, UV/S2O82− (persulfate) and Fe2+/UV to destroy recalcitrant organic compounds through the production of hydroxyl radicals. The effectiveness of AOP treatment on organic compounds is greatly dependent on the water quality of the treated water and the minimization of interference compounds. Research to date shown that AOP can effectively remove 10 to 50 percent of PFOS and less than 10 percent of PFOA from drinking water sources.
EMERGING TECHNOLOGIES
In addition to certain traditional drinking water treatment technologies, new and novel technologies in development such as chemical oxidation, ozofractionation, and sonolysis show promise for removing PFAS but have not been significantly researched for PFAS removal from drinking water. Many of these technologies may be more suitable for groundwater remediation and/or special treatment circumstances such as waste stream or concentrate treatment. Additional approaches and technologies will continue to be developed and evaluated in the future.
In recent years, technologies surrounding the treatment of PFAS have been explored and developed that go beyond full-scale processes currently employed at WTPs. Technologies that are new to the drinking water industry include chemical oxidation, ozofractionation, and sonolysis. Many emerging processes are energy intensive, and cost-effective full-scale applications have not yet been proven, making these options years away from full-scale use. Each of these processes will require additional research prior to full-scale use for PFAS removal. The use of alternative energy sources (e.g. solar energy) can help offset energy costs. Implementing any of these technologies will require an evaluation of capital and operation and maintenance impacts.
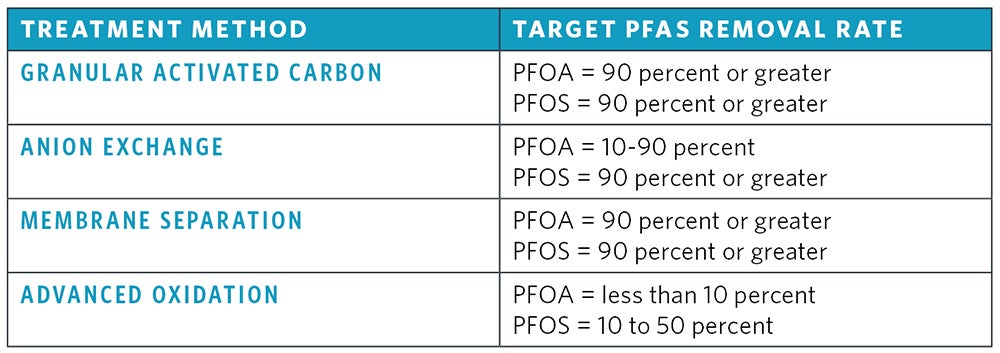
Case Study – Issaquah, Washington
Issaquah, Washington, is a Seattle-area suburb that provides drinking water to its customers using a combination of its four groundwater wells and purchased regional surface water supplies. As part of EPA’s UCMR 3, the City detected PFCs in its 250 gallon per minute Well No. 4 supply, the primary compound being PFOS. PFOS concentrations were detected at 0.472 µg/L to 0.600 µg/L in the sampling. PFOA was also detected at a level of 0.018 µg/L. In response to the PFC detections, the City shut down Well No. 4 along with the adjacent, uncontaminated 1,150 gpm Well No. 5. The Well No. 4 aquifer is above the Well No. 5 aquifer, and Well No. 5 was shut down to avoid downward PFC migration to the more productive Well No. 5 aquifer.
With the summer season quickly approaching, the City of Issaquah sought an expedited solution that would allow them to meet customer peak water demands. The City engaged HDR professionals to quickly evaluate a number of temporary and long-term treatment alternatives to eliminate the PFC contamination from Well No. 4 so Well Nos. 4 and 5 could both be returned to operation before water demands increased. For various financial, operational and water quality reasons, expanded use of purchased regional supply water was not a viable option so the City concentrated on how to return the wells to service.
As a result, we worked very closely with City and State regulators to quickly design, permit and assist construction and startup of a treatment system to successfully remove PFC contamination from Well No. 4. Features were installed so that the treatment system could be expanded to include Well No. 5 if that (lower) aquifer ever became contaminated. The installed treatment system consists of two pressure filters operated in series, each filled with 20,000 pounds of GAC. The time between City Council authorization and sending GAC-treated water to distribution was only 77 days. The treatment system has successfully reduced PFOS and PFOA concentrations to non-detectable levels in all treated water samples since its start in June 2016.
References
“Emerging Contaminants – Perfluorooctane Sulfonate (PFOS) and Perfluorooctanoic Acid (PFOA),” U.S. Environmental Protection Agency, Mar. 2014.
"Perfluorinated Chemicals (PFCs): Perfluorooctanoic Acid (PFOA) & Perfluorooctane Sulfonate (PFOS),” Association of State and Territorial Solid Waste Management Officials, 1101 17th Street, NW, Suite 707 Washington, DC 20036, Information Paper, Aug. 2015.
L. Cummings, A. Matarazzo, N. Nelson, F. Sickels, and C. Storms, “Recommendation on Perfluorinated Compound Treatment Options for Drinking Water,” New Jersey Drinking Water Quality Institute, Treatment Subcommittee, Jun. 2015.
E. Dickenson and C. Higgins, “Treatment Mitigation Strategies for Poly- and Perfluoroalkyl Substances,” Water Research Foundation, 6666 West Quincy Avenue, Denver, CO 80235, Web Report 4322, 2016.
L. Bonnette, B. J. Boros-Russo, A. Dillon, E. Apalinski, J. Berchtold, K. Fell, B. Hamill, M. Ferko, and B. Wilk, “Determination of Perfluorooctanoic Acid (PFOA) in Aqueous Samples,” New Jersey Department of Environmental Protection Division of Water Supply, Trenton, NJ, Jan. 2007.
J. A. Shoemaker, P. Grimmett, and B. Boutin, “Determination of Selected Perfluorinated Alkyl Acids in Drinking Water by Solid Phase Extraction and Liquid Chromatography/Tandem Mass Spectrometry (LC/MS/MS),” U.S. Environmental Protection Agency, Washington, DC, 2008.